Model of the alveolar region
Anna Birzle, Christian Roth and Lena Yoshihara
Efficient gas exchange requires that the blood-gas barrier has an extremely large surface area combined with a very small thickness, such that gases can pass the barrier easily. For serving this purpose, thin interior walls subdivide lung tissue into a large number of small air chambers (also known as alveoli) which are connected with the outside air through a system of branching airways.
All known mechanisms of ventilator-associated lung injuries (VALI) manifest themselves at the alveolar level of the lung, i.e. at the blood-gas barrier. In order to gain more insights into involved phenomena, we have developed a three-dimensional (3D) computational model considering the geometrical and mechanical properties of alveoli.
Artificial 3D Geometries
Non-invasive in vivo imaging techniques are mostly inapplicable to lung tissue. Hence, obtaining detailed 3D geometries of individual alveoli is very difficult. Therefore, we have developed a method for generating alveolar geometries artificially. A novel labyrinthine algorithm was proposed to generate random pathways through an assemblage of truncated octahedra representing individual alveoli. Information provided by the labyrinthine algorithm can be directly used for the generation of 3D geometries suitable for subsequent finite-element analyses.
Real 3D Geometries
Utilizing synchrotron-based X-ray tomographic microscopy, we were able to generate real and detailed 3D alveolar geometries on which we have performed finite-element simulations. This allowed us to determine, for the first time, a 3D strain state within the alveolar wall. Briefly, precision-cut lung slices, prepared from isolated rat lungs, were scanned and segmented to provide a 3D geometry. This was then discretized using newly developed tetrahedral elements. The main conclusions of this study are that the local strain in the alveolar wall can reach a multiple of the value of the global strain, for our simulations up to four times as high and that thin structures obviously cause hotspots that are especially at risk of overstretching.
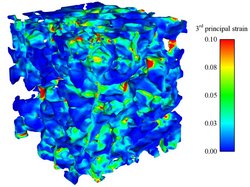
Surfactant Model
Our computational model also accounts for the alveolar liquid lining. Due to its small thickness, the main effect of the fluid film can essentially be attributed to interfacial phenomena. Hence, instead of explicitly resolving the liquid lining in the finite-element model, we consider the interfacial energy by means of equivalent surface forces applied to the tissue model.
The alveolar liquid lining is mainly composed of surface active agents, the so-called surfactant. To model the development of local surfactant concentrations and resultant surface stresses, we utilize the well-established Otis model considering adsorption, desorption, and squeeze-out processes.
Multiscale Material Model Optimization
Unfortunately, a direct experimental investigation of alveolar tissue mechanics is impossible. Therefore, we have developed a procedure to deduce material properties of individual alveolar walls from experiments with living lung parenchyma strips. Our approach is based on the combination of multiscale material models and inverse analysis techniques. Briefly, the material model on the alveolar level is iteratively adapted such that the simulated global behavior of the parenchyma strip matches the experimental results optimally.
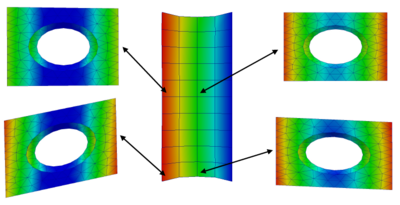
Modeling of Alveolar Gas Exchange
Simulating the exchange of oxygen and carbon dioxide between blood and air in the lung is very important in many areas of pulmonary medicine, e.g. for obtaining optimal blood oxygenation during mechanical ventilation. We are developing a novel combined FSI and multi-field scalar transport model. This model enables us to consider the influence of tissue deformation, air and blood flow on the transport and exchange of gases in the alveolus.
Publications
Please find publications on all aspects of our alveolar model here.